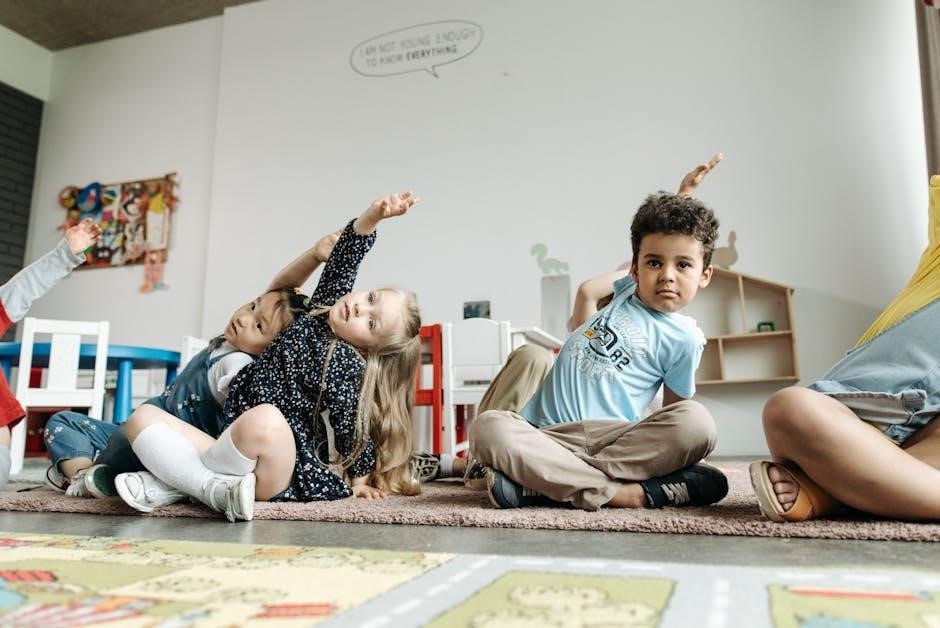
thermochemistry questions and answers pdf
Thermochemistry Questions and Answers PDF: A Comprehensive Guide
Embark on a journey through the world of thermochemistry with our comprehensive guide! This resource provides invaluable questions and answers, perfect for students and professionals seeking to deepen their understanding of energy transfer and chemical reactions.
Thermochemistry, a captivating branch of chemistry, delves into the intricate relationship between chemical reactions and energy changes. It explores the heat absorbed or released during chemical processes, providing a framework for understanding energy transformations in the chemical world. Grasping thermochemical principles is crucial for various applications, from designing efficient engines to developing new materials.
This comprehensive guide to thermochemistry questions and answers will serve as your roadmap through this fascinating subject. Whether you’re a student tackling homework problems or a professional seeking to refresh your knowledge, this resource offers a wealth of practice problems, detailed solutions, and clear explanations. Expect to see problems on the following topics: energy transfers in systems, state functions vs. path functions, types of systems (isolated, closed, open), endothermic vs. exothermic processes, enthalpy, heat capacity, calorimetry, bond energies, and thermochemical equations.
Prepare to embark on a journey of discovery, where you’ll unravel the mysteries of heat, energy, and chemical reactions. Master these concepts, and you’ll unlock a deeper appreciation for the fundamental forces that govern our universe.
Key Thermochemical Concepts
Before diving into problem-solving, it’s essential to grasp the fundamental concepts that underpin thermochemistry. Let’s begin with the definition of energy, the capacity to do work or transfer heat. In thermochemistry, we often deal with two forms of energy: kinetic energy, the energy of motion, and potential energy, the energy stored within a system.
The system and surroundings are also very important, the system is the specific part of the universe that is of interest to us, while the surroundings encompass everything else. Understanding the types of systems like isolated, closed, and open is crucial.
Heat (q) and work (w) are two primary ways energy is transferred between a system and its surroundings. Heat is the transfer of thermal energy, while work involves energy transfer through a force acting over a distance.
Familiarize yourself with these concepts, as they form the bedrock of thermochemical calculations. Keep in mind the sign conventions for heat and work (+q indicates heat absorbed by the system, -q indicates heat released, +w indicates work done on the system, and -w indicates work done by the system).
Enthalpy and its Significance
Enthalpy, denoted by the symbol H, is a thermodynamic property of a system and is defined as the sum of the system’s internal energy (U) and the product of its pressure (P) and volume (V): H = U + PV. Enthalpy is a state function, meaning its value depends only on the current state of the system, not on the path taken to reach that state.
The change in enthalpy (ΔH) is particularly useful in thermochemistry because it represents the heat absorbed or released during a chemical reaction at constant pressure, a common condition in many laboratory settings. A negative ΔH indicates an exothermic reaction (heat is released), while a positive ΔH indicates an endothermic reaction (heat is absorbed).
The standard enthalpy change (ΔH°) refers to the enthalpy change when a reaction is carried out under standard conditions (298 K and 1 atm). Standard enthalpies of formation (ΔHf°) are essential for calculating enthalpy changes of reactions using Hess’s Law.
Calorimetry: Measuring Heat Changes
Calorimetry is the experimental technique used to measure the heat exchanged during a chemical or physical process. The device used to measure this heat exchange is called a calorimeter. The fundamental principle behind calorimetry is the conservation of energy: the heat released or absorbed by the reaction is equal to the heat absorbed or released by the calorimeter and its contents.
There are two main types of calorimeters: constant-pressure calorimeters (such as coffee-cup calorimeters) and constant-volume calorimeters (bomb calorimeters). In a constant-pressure calorimeter, the heat exchanged is equal to the enthalpy change (ΔH), while in a constant-volume calorimeter, the heat exchanged is equal to the internal energy change (ΔU).
Calorimetry involves measuring the temperature change (ΔT) of a known mass of a substance (usually water) inside the calorimeter. By using the specific heat capacity (c) of the substance and the equation q = mcΔT, the heat (q) absorbed or released can be calculated. Careful calibration and insulation are crucial for accurate calorimetry measurements.
Hess’s Law and its Applications
Hess’s Law states that the enthalpy change for a chemical reaction is independent of the pathway taken between the initial and final states. In simpler terms, if a reaction can be carried out in multiple steps, the sum of the enthalpy changes for each step will equal the enthalpy change for the overall reaction.
This law is incredibly useful because it allows us to calculate enthalpy changes for reactions that are difficult or impossible to measure directly. By manipulating known thermochemical equations (reversing them or multiplying them by coefficients) and then adding them together, we can construct a pathway that leads to the desired reaction. The enthalpy change for the desired reaction is then the sum of the enthalpy changes for the manipulated equations.
Applications of Hess’s Law include determining the enthalpy changes for combustion reactions, formation reactions, and other complex chemical processes. It’s a fundamental tool in thermochemistry, enabling predictions and calculations of energy changes in a wide range of chemical reactions.
Bond Energies and Thermochemical Calculations
Bond energy, also known as bond enthalpy, represents the energy required to break one mole of a particular bond in the gaseous phase. These values are typically average values, as the energy required to break a bond can vary slightly depending on the molecular environment.
Bond energies can be used to estimate enthalpy changes (ΔH) for reactions, particularly when experimental data is unavailable. The principle behind this calculation is that breaking bonds requires energy (endothermic, positive ΔH), while forming bonds releases energy (exothermic, negative ΔH). The overall enthalpy change is approximated by summing the energies of bonds broken in the reactants and subtracting the sum of the energies of bonds formed in the products.
The formula used is: ΔH ≈ Σ (Bond energies of reactants) ⎻ Σ (Bond energies of products). Note that this calculation provides an estimate, as it uses average bond energies and neglects intermolecular forces and phase changes. Despite these limitations, bond energy calculations offer a valuable tool for predicting the exothermic or endothermic nature of a reaction and approximating its enthalpy change.
Thermochemical Equations: Representation and Interpretation
Thermochemical equations are balanced chemical equations that explicitly include the enthalpy change (ΔH) for the reaction. This value indicates the amount of heat absorbed or released during the reaction at a specified temperature and pressure, typically standard conditions (298 K and 1 atm). The sign of ΔH is crucial: a negative ΔH signifies an exothermic reaction (heat released), while a positive ΔH indicates an endothermic reaction (heat absorbed).
Coefficients in thermochemical equations represent moles, not individual molecules. Therefore, the enthalpy change is directly proportional to the amount of reactants or products involved. For example, doubling the coefficients also doubles the ΔH value. It is essential to specify the physical states (solid, liquid, gas, aqueous) of all reactants and products, as these states influence the enthalpy change.
Furthermore, reversing a thermochemical equation changes the sign of ΔH. If a reaction is exothermic in the forward direction, it will be endothermic in the reverse direction, and vice versa. Thermochemical equations provide a concise and informative way to represent chemical reactions and their associated energy changes.
State Functions vs. Path Functions
In thermodynamics, state functions are properties that depend only on the initial and final states of a system, irrespective of the path taken to achieve that change. Examples of state functions include internal energy (U), enthalpy (H), entropy (S), and Gibbs free energy (G). The change in a state function is determined solely by the difference between its final and initial values, making calculations straightforward and predictable.
In contrast, path functions are properties that depend on the process or pathway by which a change occurs. Heat (q) and work (w) are classic examples of path functions. The amount of heat exchanged or work done during a process varies depending on how the process is carried out, making their values path-dependent.
Understanding the distinction between state and path functions is crucial in thermochemistry. State functions simplify calculations of thermodynamic changes, as their values are independent of the specific steps involved. Path functions, however, require careful consideration of the process details to determine accurate values. Recognizing this difference enables precise analysis of energy changes in chemical and physical processes.
Endothermic vs. Exothermic Processes: Identifying and Differentiating
Chemical reactions and physical changes involve either the absorption or release of heat energy; These processes are categorized as either endothermic or exothermic, depending on the direction of heat flow. Understanding the difference between these two types of processes is fundamental to thermochemistry.
Endothermic processes absorb heat from their surroundings. As a result, the temperature of the surroundings decreases, and the enthalpy change (ΔH) for the process is positive (ΔH > 0). Examples of endothermic processes include melting ice, boiling water, and many chemical reactions such as the dissolution of ammonium nitrate in water.
Exothermic processes, conversely, release heat into their surroundings. The temperature of the surroundings increases, and the enthalpy change (ΔH) for the process is negative (ΔH < 0). Common examples of exothermic processes include combustion reactions, such as burning wood or natural gas, and the formation of chemical bonds.
Identifying whether a process is endothermic or exothermic is crucial for predicting the energy changes and temperature effects associated with it. By examining the enthalpy change (ΔH), one can readily determine whether a reaction requires energy input or releases energy into the environment.
Specific Heat Capacity and its Role in Calculations
Specific heat capacity (c) is a fundamental property of a substance that quantifies the amount of heat required to raise the temperature of one gram of the substance by one degree Celsius (or one Kelvin). It is an intensive property, meaning it does not depend on the amount of substance present.
Different substances have different specific heat capacities due to variations in their molecular structure and intermolecular forces. For example, water has a relatively high specific heat capacity (4.184 J/g°C), which means it can absorb a significant amount of heat without a large temperature change. Metals, on the other hand, generally have lower specific heat capacities.
Specific heat capacity plays a crucial role in calorimetry calculations, where heat transfer between objects is measured. The amount of heat (q) transferred can be calculated using the formula: q = mcΔT, where m is the mass of the substance, c is its specific heat capacity, and ΔT is the change in temperature.
By knowing the specific heat capacities of the substances involved, we can accurately determine the amount of heat gained or lost during a process, allowing us to analyze and understand the energy changes in chemical reactions and physical transformations.
Solving Thermochemistry Problems: Step-by-Step Approach
Tackling thermochemistry problems can seem daunting, but a systematic approach can simplify the process. First, carefully read the problem statement and identify what is being asked. Highlight key information, such as given values for enthalpy changes, masses, specific heat capacities, and temperatures.
Next, determine the relevant thermochemical concepts and equations needed to solve the problem. This may involve applying Hess’s Law, using the heat capacity formula (q = mcΔT), or working with standard enthalpies of formation. Write down the equations you plan to use.
Organize the given information and convert all values to consistent units (e.g., Joules for energy, grams for mass, and Celsius or Kelvin for temperature). Draw a diagram or flowchart to visualize the steps involved in the process, if helpful.
Substitute the known values into the appropriate equations and solve for the unknown variable. Pay close attention to signs (positive for endothermic, negative for exothermic). Finally, check your answer for reasonableness and ensure it has the correct units. If necessary, review your steps to identify any errors.
Practice Problems with Detailed Solutions
Sharpen your thermochemistry skills with a curated collection of practice problems, each accompanied by a detailed, step-by-step solution. These problems cover a wide range of thermochemical concepts, including calorimetry, enthalpy changes, Hess’s Law, and bond energies. Work through each problem independently, applying the problem-solving strategies discussed earlier.
After attempting a problem, carefully compare your solution to the detailed solution provided. Pay close attention to the reasoning behind each step, the correct application of formulas, and the proper handling of units and signs. Identify any areas where you struggled and review the relevant concepts.
These practice problems are designed to reinforce your understanding of thermochemistry and build your confidence in tackling challenging questions. By working through a variety of examples, you’ll develop a stronger intuition for how thermochemical principles apply in different contexts.
Remember, practice is key to mastering any scientific discipline. Use these problems as an opportunity to hone your skills and solidify your understanding of thermochemistry.
Common Mistakes to Avoid in Thermochemistry Calculations
Thermochemistry calculations can be tricky, and it’s easy to fall into common traps. One frequent error is incorrect unit conversions; always ensure consistency in units (Joules vs. Kilojoules, grams vs. kilograms) before plugging values into equations. Another pitfall is mishandling signs; remember that exothermic reactions have negative enthalpy changes, while endothermic reactions have positive ones.
Forgetting to balance chemical equations before using them in calculations is another common mistake. A balanced equation is crucial for determining the correct stoichiometric ratios between reactants and products. Moreover, students often confuse heat capacity and specific heat capacity, using the wrong value in calorimetry calculations.
When applying Hess’s Law, ensure you manipulate enthalpy changes correctly when reversing or multiplying reactions. Always double-check that you’re summing the enthalpy changes of the individual steps in the correct direction. Finally, be careful with bond energies; remember that bond energies are always positive, representing the energy required to break a bond.
By being mindful of these common mistakes, you can significantly improve your accuracy in thermochemistry calculations.